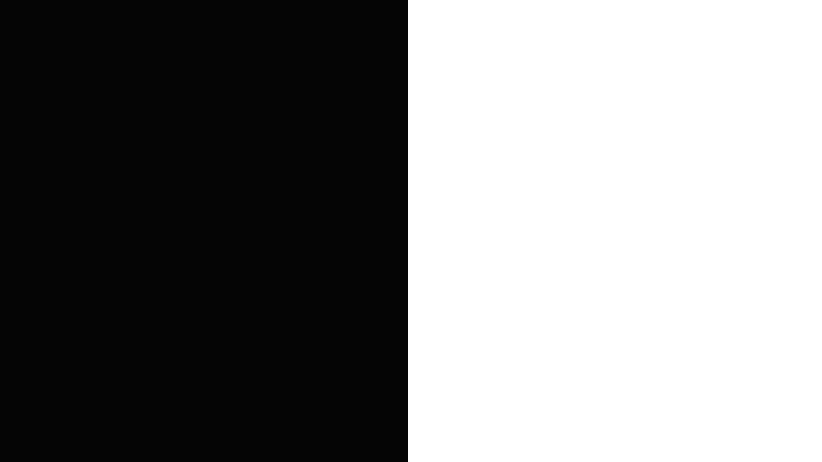
+2.65
Perceptual adaptation
Perceptual adaptation is a unique function of the brain that accounts for the differences viewed in the world, as it relates to the senses. This phenomenon occurs in all senses, including vision, hearing, touch, and smell. An example is when images sensed through the eyes are relayed to the visual cortex of the brain, and if vision is altered slightly, the brain accounts for the difference and will allow one to perceive the world as "normal." This is a compensation mechanism the brain uses for the world to appear normal in our minds when our world has obviously been altered from its regular state.[1] This is an important aspect in potential alterations to the visual field, effectiveness in compensating for alterations in the visual field, visual adaptation, and face recognition.
Experimental support
In the 1890s, psychologist George M. Stratton conducted experiments in which he tested the theory of perceptual adaptation. In one experiment, he wore a reversing glasses for 21½ hours over three days, with no change in his vision. After removing the glasses, "normal vision was restored instantaneously and without any disturbance in the natural appearance or position of objects."
Modern version of inverting mirrors with harness.
On a later experiment, Stratton wore the glasses for eight whole days. By day four, the images seen through the instrument were still upside down. However, on day five, images appeared upright until he concentrated on them; then they became inverted again. By having to concentrate on his vision to turn it upside down again, especially when he knew images were hitting his retinas in the opposite orientation as normal, Stratton deduced his brain had reprocessed his vision and adapted to the changes in vision.
Potential alterations to the visual field
The visual field can be altered in many ways. The various ways that it can be altered is by angles, or by orientation. Optometrists alter the visual field by altering angles (i.e. fashion the subject with glasses that construe the visual field by differing angles). George Stratton conducted experiments where the visual field was altered by an angle of forty five degrees and his brain was able to adapt to the change and perceive the world as normal. Also, the field can be altered making the subject see the world upside down. But, as the brain adjusts to the change, the world appears "normal."
Effectiveness in compensating for alterations in the visual field
An altered visual field does not adversely affect one’s life. Our perceptual adaptation allows us to adapt to the world and live life as normal. Therefore, with an altered visual field, the limits are nonexistent. One is able to do whatever they please without limitations. In some extreme experiments, psychologists have tested to see if a pilot can fly a plane with altered vision. All of the pilots that were fitted with the goggles that alter their vision were able to safely navigate the aircraft with ease.
Visual Adaptation
Visual Adaptation is defined as the aftereffects of exposure to a certain visual stimulus or visual pattern that caused one to lose sensitivity to that pattern and engage in stimulus bias. An example of this phenomenon is the "lilac chaser", introduced by Jeremy Hinton. The stimulus here are lilac circles, that once removed, leave green circles that then become the most prominent stimulus. The fading of the lilac circles is due to a loss of sensitivity to that stimulus and the adaptation to the new stimulus. To experience the "lilac chaser" effect, the subject needs to fixate their eyes on the cross in the middle of the image, and after a while the effect will settle in. Visual coding, a process involved in visual adaptation, is the means by which the brain adapts to certain stimuli, resulting in a biased perception of those stimuli. This phenomenon is referred to as visual plasticity; the brain's ability to change and adapt according to certain, repeated stimuli, altering the way information is perceived and processed.
Lilac Chaser from Jeremy Hinton's experiment
The rate and strength of visual adaptation depends heavily on the number of stimuli presented simultaneously, as well as the amount of time for which the stimulus is present. Visual adaptation was found to be weaker when there were more stimuli present, than when the stimuli were only a few. Moreover, studies have found that stimuli can rival each other, which explains why higher numbers of simultaneous stimuli lead to lower stimulus adaptation. Studies have also found that visual adaptation can have a reversing effect; if the stimulus is absent long enough, the aftereffects of visual adaptation will subside. Lastly, studies have also shown that visual adaptation occurs in the early stages of processing.
Face Recognition
Perceptual adaptation plays a big role in identifying faces. The phenomenon of aftereffects applies to faces as well. In an experiment conducted by Gillian Rhodes, the impact of face adaptation was investigated, along with whether visual adaptation has an impact on recognizing faces. The experiment found that perceptual adaptation does, in fact, have an impact on face recognition. Individuals tend to adapt to common facial features as early as after five minutes of looking at them. This suggests that humans adapt to common facial features, leaving neural resources and space to identify uncommon characteristics and features, which is how humans identify specific faces on a case-by-case basis.
Perceptual aftereffects for face recognition occur for several different stimuli, including gender, ethnicity, identity, emotion, and attractiveness of a face. The fact that this distinction occurs, implies that face recognition is a process that happens on a higher level and later on in the visual encoding, rather than early on within visual adaptation. The fact that the aftereffects in face recognition in particular are so strong, suggests that it is for the purpose of regulation of how processes work. This provides a sense of constancy in an individual's perception, while adapting to differences and possible versions of a stimulus allows for constancy and stability, and makes it easier to adapt to variations in a stimulus, while recognizing commonalities. These face perception cues are encoded in an individual's brain for extended periods of time, ensuring consistency over the individual's lifespan. A young person would perceive stimuli the same way as the an older individual.
Auditory Adaptation
Auditory adaptation, as perceptual adaptation with other senses, is the process by which individuals adapt to sounds and noises. As research has shown, as time progresses, individuals tend to adapt to sounds and tend to distinguish them less frequently after a while. Sensory adaptation tends to blend sounds into one, variable sound, rather than having several separate sounds as a series. Moreover, after repeated perception, individuals tend to adapt to sounds to the point where they no longer consciously perceive it, or rather, "block it out". An individual that lives close to the train tracks, will eventually stop noticing the sounds of passing trains. Similarly, individuals living in larger cities no longer notice traffic sounds after a while. Moving to a completely different area, such as a quiet countryside, would then be aware of the silence, crickets, etc.
Other Types of Sensory Adaptation
Perceptual adaptation is a phenomenon that occurs for all of the senses, including smell and touch. An individual can adapt to a certain smell with time. Smokers, or individuals living with smokers, tend to stop noticing the smell of cigarettes after some time, whereas people not exposed to smoke on a regular basis will notice the smell instantly. The same phenomenon can be observed with other types of smell, such as perfume, flowers, etc. The human brain can distinguish smells that are unfamiliar to the individual, while adapting to those it is used to and no longer require to be consciously recognized. This phenomenon also applies to the sense of touch. An unfamiliar piece of clothing that was just put on will be noticed instantly; however, once it has been worn for a while, the mind will adapt to its texture and ignore the stimulus.
Subjectivity of color perception
Nothing categorically distinguishes the visible spectrum of electromagnetic radiation from invisible portions of the broader spectrum. In this sense, color is not a property of electromagnetic radiation, but a feature of visual perception by an observer. Furthermore, there is an arbitrary mapping between wavelengths of light in the visual spectrum and human experiences of color. Although most people are assumed to have the same mapping, the philosopher John Lockerecognized that alternatives are possible, and described one such hypothetical case with the "inverted spectrum" thought experiment. For example, someone with an inverted spectrum might experience green while seeing 'red' (700 nm) light, and experience red while seeing 'green' (530 nm) light. Synesthesia (or ideasthesia) provides some atypical but illuminating examples of subjective color experience triggered by input that is not even light, such as sounds or shapes. The possibility of a clean dissociation between color experience from properties of the world reveals that color is a subjective psychological phenomenon.
The Himba people have been found to categorize colors differently from most Euro-Americans and are able to easily distinguish close shades of green, barely discernible for most people.[23] The Himba have created a very different color scheme which divides the spectrum to dark shades (zuzu in Himba), very light (vapa), vivid blue and green (buru) and dry colors as an adaptation to their specific way of life.
Perception of color depends heavily on the context in which the perceived object is presented. For example, a white page under blue, pink, or purple light will reflect mostly blue, pink, or purple light to the eye, respectively; the brain, however, compensates for the effect of lighting (based on the color shift of surrounding objects) and is more likely to interpret the page as white under all three conditions, a phenomenon known as color constancy.
Theory of colour, Johannes Itten
Johannes Itten (11 November 1888 – 25 March 1967) was a Swiss expressionist painter, designer, teacher, writer and theorist associated with the Bauhaus (Staatliche Bauhaus) school. Together with German-American painter Lyonel Feininger and German sculptor Gerhard Marcks, under the direction of German architect Walter Gropius, Itten was part of the core of the Weimar Bauhaus.
http://monoskop.org/images/4/46/Itten_Johannes_The_Elements_of_Color.pdf
![]() Daler-Rowney-Colour-wheel-W.jpg |
---|
![]() tumbling-E1.jpg |
![]() PastedGraphic-1.png |
![]() 300px-Kanizsa_triangle.svg.png |
![]() 330px-Numerosityadaptation.png |
![]() chapter6-42-728.jpg |
![]() color_blindness_test3.png |
![]() fig7.gif |
![]() eye-charts-358x338.gif |
![]() artists-color-wheel.jpg |
![]() insidethelines.jpg |
![]() fig12.gif |
![]() PSM_V54_D326_Optical_illusion_image_used_in_psychological_tests.png |
VISUAL PERCEPTION & EYESIGHT
Visual perception is the ability to interpret the surrounding environment by processing information that is contained in visible light. The resulting perception is also known as eyesight, sight, or vision (adjectival form: visual, optical, or ocular). The various physiological components involved in vision are referred to collectively as the visual system, and are the focus of much research in psychology, cognitive science, neuroscience, and molecular biology, collectively referred to as vision science.
The visual system in animals allows individuals to assimilate information from their surroundings. The act of seeing starts when the lens of the eye focuses an image of its surroundings onto a light-sensitive membrane in the back of the eye, called the retina. The retina is actually part of the brain that is isolated to serve as a transducer for the conversion of patterns of light into neuronal signals. The lens of the eye focuses light on the photoreceptive cells of the retina, which detect the photons of light and respond by producing neural impulses. These signals are processed in a hierarchical fashion by different parts of the brain, from the retina upstream to central ganglia in the brain.
Note that up until now much of the above paragraph could apply to octopi, mollusks, worms, insects and things more primitive; anything with a more concentrated nervous system and better eyes than say a jellyfish. However, the following applies to mammals generally and birds (in modified form): The retina in these more complex animals sends fibers (the optic nerve) to the lateral geniculate nucleus, to the primary and secondary visual cortex of the brain. Signals from the retina can also travel directly from the retina to the superior colliculus.
The perception of objects and the totality of the visual scene is accomplished by the visual association cortex. The visual association cortex combines all sensory information perceived by the striate cortex which contains thousands of modules that are part of modular neural networks. The neurons in the striate cortex send axons to the extrastriate cortex, a region in the visual association cortex that surrounds the striate cortex
COLOUR VISION
Color vision is the ability of an organism or machine to distinguish objects based on the wavelengths (or frequencies) of the light they reflect, emit, or transmit. Colors can be measured and quantified in various ways; indeed, a person's perception of colors is a subjective process whereby the brain responds to the stimuli that are produced when incoming light reacts with the several types of cone cells in the eye. In essence, different people see the same illuminated object or light source in different ways.
Wavelength and hue detection
Isaac Newton discovered that white light splits into its component colors when passed through a dispersive prism. Newton also found that he could recombine these colors by passing them through a different prism to make white light.
The characteristic colors are, from long to short wavelengths (and, correspondingly, from low to high frequency), red, orange, yellow, green, cyan, blue, and violet. Sufficient differences in wavelength cause a difference in the perceived hue; the just-noticeable difference in wavelength varies from about 1 nm in the blue-green and yellow wavelengths, to 10 nm and more in the longer red and shorter blue wavelengths. Although the human eye can distinguish up to a few hundred hues, when those pure spectral colors are mixed together or diluted with white light, the number of distinguishable chromaticities can be quite high.
In very low light levels, vision is scotopic: light is detected by rod cells of the retina. Rods are maximally sensitive to wavelengths near 500 nm, and play little, if any, role in color vision. In brighter light, such as daylight, vision is photopic: light is detected by cone cells which are responsible for color vision. Cones are sensitive to a range of wavelengths, but are most sensitive to wavelengths near 555 nm. Between these regions, mesopic vision comes into play and both rods and cones provide signals to the retinal ganglion cells. The shift in color perception from dim light to daylight gives rise to differences known as the Purkinje effect.
The perception of "white" is formed by the entire spectrum of visible light, or by mixing colors of just a few wavelengths, such as red, green, and blue, or by mixing just a pair of complementary colors such as blue and yellow
Theories of color vision
Two complementary theories of color vision are the trichromatic theory and the opponent process theory. The trichromatic theory, or Young–Helmholtz theory, proposed in the 19th century by Thomas Young and Hermann von Helmholtz, as mentioned above, states that the retina's three types of cones are preferentially sensitive to blue, green, and red. Ewald Hering proposed the opponent process theory in 1872. It states that the visual system interprets color in an antagonistic way: red vs. green, blue vs. yellow, black vs. white. Both theories are now accepted as valid, describing different stages in visual physiology, visualized in the diagram on the right. Green ←→ Magenta and Blue ←→ Yellow are scales with mutually exclusive boundaries. In the same way that there cannot exist a "slightly negative" positive number, a single eye cannot perceive a blueish-yellow or a reddish-green. (But such impossible colors can be perceived due to binocular rivalry.)
Cone cells in the human eye
Cone typeNameRangePeak wavelength:
S β 400–500 nm420–440 nm
M γ 450–630 nm534–555 nm
L ρ 500–700 nm564–580 nm
A range of wavelengths of light stimulates each of these receptor types to varying degrees. Yellowish-green light, for example, stimulates both L and M cones equally strongly, but only stimulates S-cones weakly. Red light, on the other hand, stimulates L cones much more than M cones, and S cones hardly at all; blue-green light stimulates M cones more than L cones, and S cones a bit more strongly, and is also the peak stimulant for rod cells; and blue light stimulates S cones more strongly than red or green light, but L and M cones more weakly. The brain combines the information from each type of receptor to give rise to different perceptions of different wavelengths of light.
The opsins (photopigments) present in the L and M cones are encoded on the X chromosome; defective encoding of these leads to the two most common forms of color blindness. The OPN1LW gene, which codes for the opsin present in the L cones, is highly polymorphic (a recent study by Verrelli and Tishkoff found 85 variants in a sample of 236 men). A very small percentage of women may have an extra type of color receptor because they have different alleles for the gene for the L opsin on each X chromosome. X chromosome inactivation means that only one opsin is expressed in each cone cell, and some women may therefore show a degree of tetrachromatic color vision. Variations in OPN1MW, which codes the opsin expressed in M cones, appear to be rare, and the observed variants have no effect on spectral sensitivity.
![]() 1Mcolors.png |
---|
![]() 540px-Cone-fundamentals-with-srgb-spectrum.svg.png |
![]() 600px-Ventral-dorsal_streams.svg.png |
![]() 669px-Eyesensitivity.svg.png |
![]() Modern_Color_Vision_Model.svg.png |
![]() Spectrum_locus_12.png |
Color processing
Color processing begins at a very early level in the visual system (even within the retina) through initial color opponent mechanisms. Both Helmholtz's trichromatic theory, and Hering's opponent process theory are therefore correct, but trichromacy arises at the level of the receptors, and opponent processes arise at the level of retinal ganglion cells and beyond. In Hering's theory opponent mechanisms refer to the opposing color effect of red–green, blue–yellow, and light–dark. However, in the visual system, it is the activity of the different receptor types that are opposed. Some midget retinal ganglion cells oppose L and M cone activity, which corresponds loosely to red–green opponency, but actually runs along an axis from blue-green to magenta. Small bistratified retinal ganglion cells oppose input from the S cones to input from the L and M cones. This is often thought to correspond to blue–yellow opponency, but actually runs along a color axis from lime green to violet.
Visual information is then sent to the brain from retinal ganglion cells via the optic nerve to the optic chiasma: a point where the two optic nerves meet and information from the temporal (contralateral) visual field crosses to the other side of the brain. After the optic chiasma the visual tracts are referred to as the optic tracts, which enter the thalamus to synapse at the lateral geniculate nucleus (LGN)
The lateral geniculate nucleus (LGN) is divided into laminae (zones), of which there are three types: the M-laminae, consisting primarily of M-cells, the P-laminae, consisting primarily of P-cells, and the koniocellular laminae. M- and P-cells receive relatively balanced input from both L- and M-cones throughout most of the retina, although this seems to not be the case at the fovea, with midget cells synapsing in the P-laminae. The koniocellular laminae receive axons from the small bistratified ganglion cells.
After synapsing at the LGN, the visual tract continues on back to the primary visual cortex (V1) located at the back of the brain within the occipital lobe. Within V1 there is a distinct band (striation). This is also referred to as "striate cortex", with other cortical visual regions referred to collectively as "extrastriate cortex". It is at this stage that color processing becomes much more complicated.
In V1 the simple three-color segregation begins to break down. Many cells in V1 respond to some parts of the spectrum better than others, but this "color tuning" is often different depending on the adaptation state of the visual system. A given cell that might respond best to long wavelength light if the light is relatively bright might then become responsive to all wavelengths if the stimulus is relatively dim. Because the color tuning of these cells is not stable, some believe that a different, relatively small, population of neurons in V1 is responsible for color vision. These specialized "color cells" often have receptive fields that can compute local cone ratios. Such "double-opponent" cells were initially described in the goldfish retina by Nigel Daw; their existence in primates was suggested by David H. Hubel and Torsten Wiesel and subsequently proven by Bevil Conway. As Margaret Livingstone and David Hubel showed, double opponent cells are clustered within localized regions of V1 called blobs, and are thought to come in two flavors, red–green and blue–yellow. Red–green cells compare the relative amounts of red–green in one part of a scene with the amount of red–green in an adjacent part of the scene, responding best to local color contrast (red next to green). Modeling studies have shown that double-opponent cells are ideal candidates for the neural machinery of color constancy explained by Edwin H. Land in his retinex theory.
From the V1 blobs, color information is sent to cells in the second visual area, V2. The cells in V2 that are most strongly color tuned are clustered in the "thin stripes" that, like the blobs in V1, stain for the enzyme cytochrome oxidase (separating the thin stripes are interstripes and thick stripes, which seem to be concerned with other visual information like motion and high-resolution form). Neurons in V2 then synapse onto cells in the extended V4. This area includes not only V4, but two other areas in the posterior inferior temporal cortex, anterior to area V3, the dorsal posterior inferior temporal cortex, and posterior TEO. Area V4 was initially suggested by Semir Zeki to be exclusively dedicated to color, but this is now thought to be incorrect. In particular, the presence in V4 of orientation-selective cells led to the view that V4 is involved in processing both color and form associated with color. Color processing in the extended V4 occurs in millimeter-sized color modules called globs. This is the first part of the brain in which color is processed in terms of the full range of hues found in color space.
Anatomical studies have shown that neurons in extended V4 provide input to the inferior temporal lobe . "IT" cortex is thought to integrate color information with shape and form, although it has been difficult to define the appropriate criteria for this claim. Despite this murkiness, it has been useful to characterize this pathway (V1 > V2 > V4 > IT) as the ventral stream or the "what pathway", distinguished from the dorsal stream ("where pathway") that is thought to analyze motion, among many other features.
.